What to do with wet waste
- Chloe Coates
- Aug 1, 2024
- 9 min read
Updated: Aug 2, 2024
This article explores the untapped potential of waste water and wet waste - byproducts of our societal and industrial activities that have long been viewed as problematic refuse. From sewage to industrial effluents, these waste streams contain valuable resources that, if properly harnessed, could contribute to decarbonisation.
What do we mean by waste water and wet waste?
Wastewater refers to sources of waste water including sewage, industrial waste water streams (including from breweries, dairy processing, wine production, pulp and paper production, etc.) and animal waste like manure. Food waste and certain municipal solid waste in landfills could also be considered wet waste because of their high moisture content. We are essentially referring to a subset of organic (largely carbon-containing) waste streams, whose high water content makes them more amenable to water-based processing.
These waste streams are a by-product of our society and need to be treated before being returned to waterways and the natural environment, to avoid damaging ecosystems and food production ecosystems. Undesired pollutants include PFAS ‘forever chemicals’, pathogens and microplastics.
What happens to waste water today?
In most of the developing world, 80% of waste water is returned to the environment without further treatment. In most developed economies there are strict regulations around treating wastewater before it can be returned to the environment, including the Drinking Water Directive in Europe and the Clean Water Act of the Environmental Protection Agency in the US.
In general, sewage undergoes several treatment steps including physical separation i.e. removal of grit and large solid waste and treatment with microbes to break down organic matter; this microbial treatment can be aerobic (with air pumped through) or anaerobic. The pumping associated with aerobic treatment is one of the largest energy demands of waste water treatment accounting for 40-75% of the total energy requirements, which could reach 730 TWh globally by 2050. Following these primary and secondary treatment steps, the waste water may undergo further treatment including with disinfectants, as well as some level of dewatering to reduce the water content and volume of the waste.
The treated water is then returned to waterways, whilst the remaining sludge (also called biosolids) can be further treated, sent to landfill, spread thinly on agricultural land (known as landspreading) or incinerated. The environmental and emissions implications of each of these choices vary:
in landfills, the organic matter is likely to decompose further to produce methane and carbon dioxide, with a large near-term warming impact from the methane in particular (GWP20 ~ 84 CO2e);
landspreading may also lead to methane emissions, with particular concerns around the implications of increasing forever chemicals, pathogens and microplastics that remain in the sludge and are thus returned to the environment;
incineration minimises waste volumes, but in the absence of carbon capture and storage, releases large amounts of CO2 into the environment.
Industrial waste streams are usually more homogeneous than sewage, but need to reduce the amount of reactive organic waste before returning the water to the environment. This is known as (bio)chemical oxygen demand (the BOD or COD) which indicates how much oxygen is required to break down this reactive organic matter (for example if a waste stream has a BOD of 1000 mg/L then 1000 mg/L of dissolved oxygen must be provided). Regulation exists that sets upper limits of what is acceptable to return to the environment. Large industrial facilities might have their own dedicated water treatment, where as others pay a third party for external treatment.
The sludge output from wastewater treatment also contains valuable organic (and inorganic) matter that could be recovered for a range of products and applications. Could we be making better use of these resources to improve both the treatment of the water, and minimise the emissions associated with sludge disposal? There are existing and emerging technologies that tackle some of these problems – we explore these in the next section.
Maximising value from waste
What is the best way to decide which is the best technology path to pursue? Which is the most valuable product? Should we use the waste to produce energy, or high value chemicals, or retain the carbon atoms as much as possible for CDR? The answer is, of course, it depends.
There are many parallels here with the discussion around (solid) biomass. EIP’s Andy Lubershane has written on the topic on his blog Steel for Fuel. Likewise, Poppy Russell at Counteract explores the debate around using biomass for energy vs using it for carbon removal in her piece on BECCS vs BiCRS.
We can take a simplistic approach of determining what has the highest market value based on using 1 tonne of wastewater sludge (or MSW or manure). This depends on the energy production efficiency and how much one can sell the resulting energy for. However, as the market for CDR becomes more mature and the value of carbon removal increases, at some point the carbon content of that waste becomes more valuable than the energy content. This is known as the Aines Principle (thank you Poppy for pointing me to the concept).
It is of course possible to use the biomass for energy first and then to capture the carbon, but this introduces higher CAPEX, and also reduces the efficiency of energy (and thus value) production, and lowers the CDR efficiency at the same time. For a given energy generation process, capture process and at given prices, the balance of value will vary. The figure below is taken from an article by Woodall and McCormick where they explore these tradeoffs for a case study of using MSW for different applications.
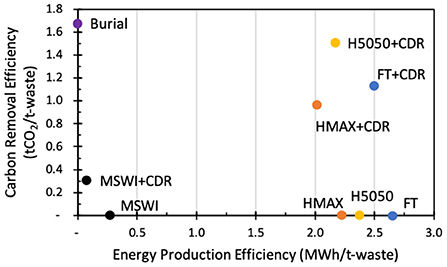
So what could we use the organic waste for?
Electricity: The energy contents of the organic waste could be used to produce electricity (either directly or indirectly via a fuel). Whilst this could displace current fossil-based generation and provide electricity with fewer emissions, the rapid cost declines of other clean energy sources like wind and solar could make them preferable from an impact perspective. Example companies include: Pipeline Organics, AquaCycl
Hydrogen: Producing hydrogen from the hydrocarbons is likely to have lower energy requirements than hydrogen from electrolysis. But the distributed nature of wet waste streams is at odds with the energy intensity and cost of transporting hydrogen. If hydrogen production from waste is coupled with carbon capture then this offers a good option for extracting the energy via hydrogen fuel, but also minimising CO2 emissions. Gasification technologies that produce syngas (a mixture of carbon monoxide and hydrogen) offer a good precursor to make many of the fossil-derived chemicals we need for our modern lives, but using a biogenic carbon source. Examples: Ki Hydrogen, BlueFlux Energy
Methane/biogas: As with making electricity – if the biogas/biomethane is just going to be used to generate electricity, or home heating, it could be argued that we have better options to decarbonise both of those activities in the form of renewables generation and heat pumps. There are new technologies that improve the efficiency of methane production in biogas (e.g. via methanogenesis) and also that increase the efficiency of methane utilisation using fuel cells rather than gas turbines. In the near term producing biomethane/biogas in developing countries for cooking, as a substitute for polluting and emissions-intensive solid cooking fuels, is a good option. Examples: WASE, Vertus Energy, Reverion
Liquid fuels (or ‘biocrude’): There is likely to be high demand liquid fuels for hard-to-abate transport sectors like aviation. There are numerous pathways for using wet biomass to make bio-crude to produce these sustainable aviation fuels (SAFs), and challenges in securing feedstocks suggest that the broader the feedstock pool, the better. The challenge is in dealing with heterogeneous inputs streams like wet waste to produce a specific desired fuel. Example: Firefly
Carbon removal: We could take the waste and designate it as carbon removal. Either by burying the contaminated waste as is (Vaulted Deep), converting it to hydrochar or biochar or crude-like oil (Charm Industrial) and burying that, or subjecting the the waste to supercritical conditions to produce a pure stream of CO2 and burying that instead. Example: Kairos Carbon

Technologies
Anaerobic digestion: the incumbent
Anaerobic digestion is a process through which bacteria break down organic matter in the absence of oxygen. The process produces biogas, which is a mixture of methane (typically 50-70%) and carbon dioxide (30-50%). The biogas can be burned directly onsite to produce heat or energy, or upgraded to produce purer (bio)methane that can be fed into the natural gas grid. Not all of the organic matter is converted into biogas, there is always a recalcitrant solid component remaining that is then used for landspreading/incineration/sent to landfill.
The chemical reactions that occur in stages during anaerobic digestion are hydrolysis, fermentation, also called acidogenesis (the formation of soluble organic compounds and short-chain organic acids), and methanogenesis (the bacterial conversion of organic acids into methane and carbon dioxide).The main challenges for anaerobic digesters are the high CAPEX and slow reaction times—on the order of days-weeks.
The market for biogas/biomethane was 35 Mtoe in 2018, which IEA projects is less than 5% of the potential market. But the costs of producing biogas are high (between $10-16/MBtu), mostly due to CAPEX amortisation costs for the digester itself. There are numerous startups working on innovations to improve digester efficiency and/or reduce residence times to improve the economics of biogas production.
Electrochemical processes: supercharging microbes
Electrochemical processes for wet waste treatment exploit the interactions of microbes with electrical current. Two approaches exist. In electrolysis mode by applying an electrical current, microbes can accelerate chemical reactions to convert the organic waste to fuels like hydrogen or methane. Or the reverse approach exists, where the chemical reactions can be used to generate an electrical current and therefore provide electricity.
Electromethanogenesis is a process where methane (CH₄) is produced from carbon dioxide (CO₂) and electricity. It is a specific type of microbial electrolysis involving a specialised group of microorganisms called methanogens, which can reduce CO₂ to methane using electrons from an electrode (the cathode). Electromethanogenesis can be exploited to make an AD reactor more productive, leading to higher yields and faster production of biomethane. Yield improvements, and AD yields generally, are highly dependent on input conditions (temperature, pH) and feedstock composition.
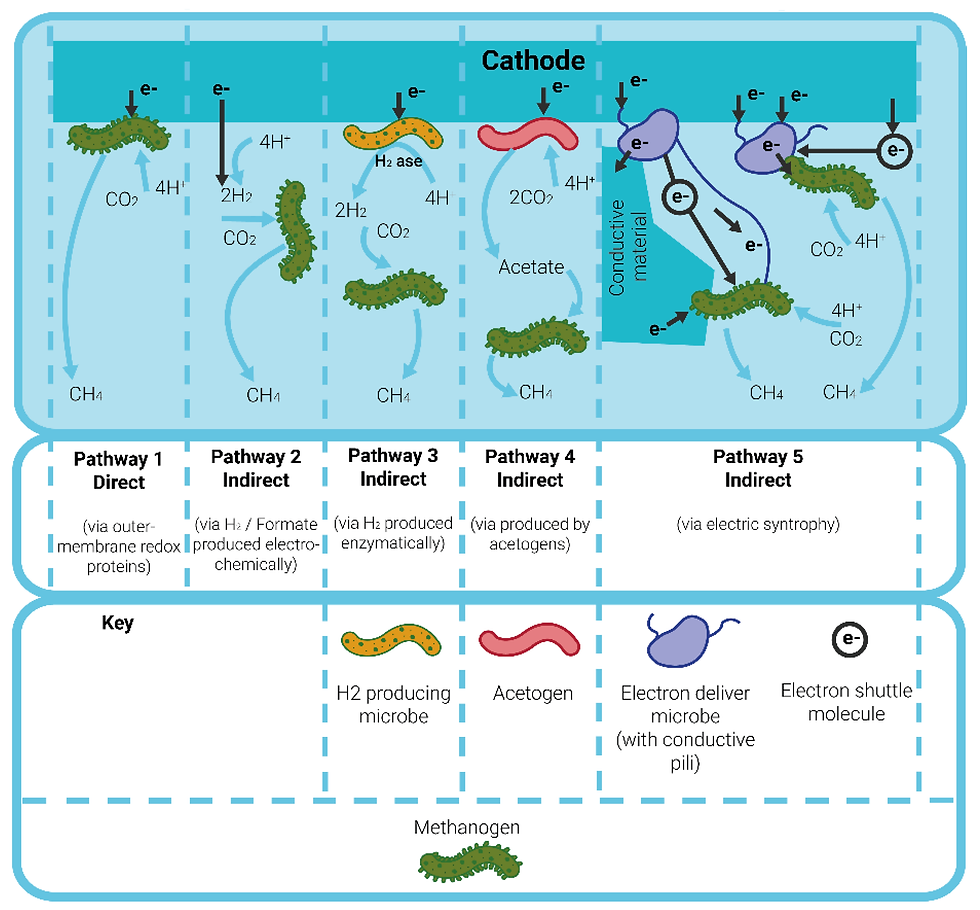
The challenge for these bio-electrochemical approaches is the sensitivity of the microbes to the input, which therefore require fairly homogeneous waste streams, as well as electrode fouling and stability.
Hydrothermal processes: wet burning
Hydrothermal processes offer advantages over traditional thermochemical processes (like pyrolysis and gasification) for wet organic waste valorisation, as they can handle high moisture content feedstocks without the need for energy-intensive drying steps. The choice of process depends on the desired product (solid, liquid, or gaseous fuel) and the specific characteristics of the wet waste feedstock.
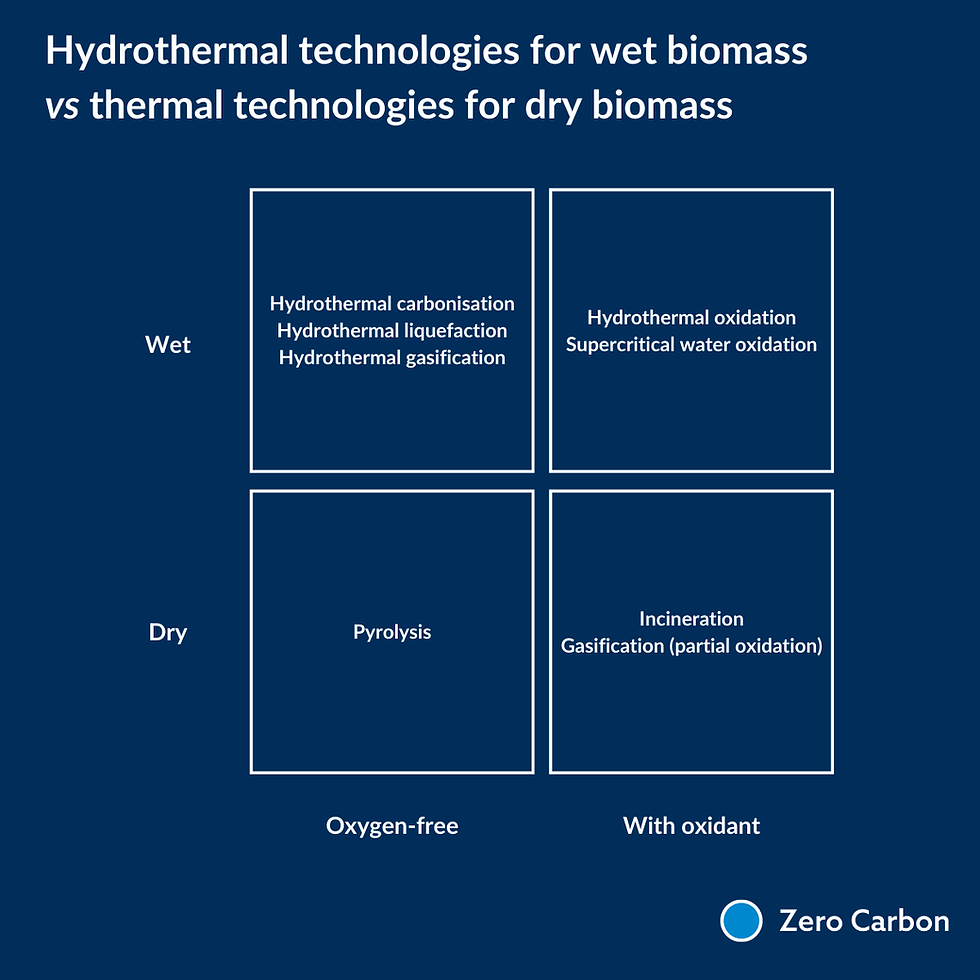
Hydrothermal Carbonisation (HTC) converts wet biomass into a coal-like solid fuel called hydrochar, with water and gases at moderate temperatures (180-350°C) and high pressure.
Hydrothermal Liquefaction (HTL) converts wet biomass into a bio-crude oil, gases and solid residues using higher temperatures (300-350°C) and high pressure.
Hydrothermal Gasification (HTG) converts wet biomass into gaseous fuels like hydrogen, methane, and carbon monoxide at high temperatures (500-700°C) and high pressure.
Hydrothermal Oxidation (HTO) converts wet biomass into ‘fermentable acids’ i.e. it breaks down the more recalcitrant material into smaller hydrocarbons, which can be more easily broken down by an anaerobic digester, increases yield and speed of anaerobic digestion process. It requires moderate temperatures and pressure, in the presence of an oxidant.
Supercritical water oxidation (SCWO) converts wet biomass into CO2 and water, and inorganic salts fall out of solution. Oxidation occurs quickly and completely under such conditions, converting even the most challenging wastes into carbon dioxide, water, minerals and heat (the oxidation of carbon-based inputs is exothermic). It requires supercriticial conditions (high temperatures of >374°C and pressures of >221 bar.) and an oxidant. Under these conditions, non-polar molecules (organic solvents, oils, resins) become completely miscible in water, but salts fall out of solution, which is pretty much the opposite of what happens in water under ambient conditions.
For all hydrothermal technologies one of the challenges to overcome is the scaling and corrosion of the reactors–a problem that becomes more acute as the conditions because more extreme.
Market map of companies working on these technologies
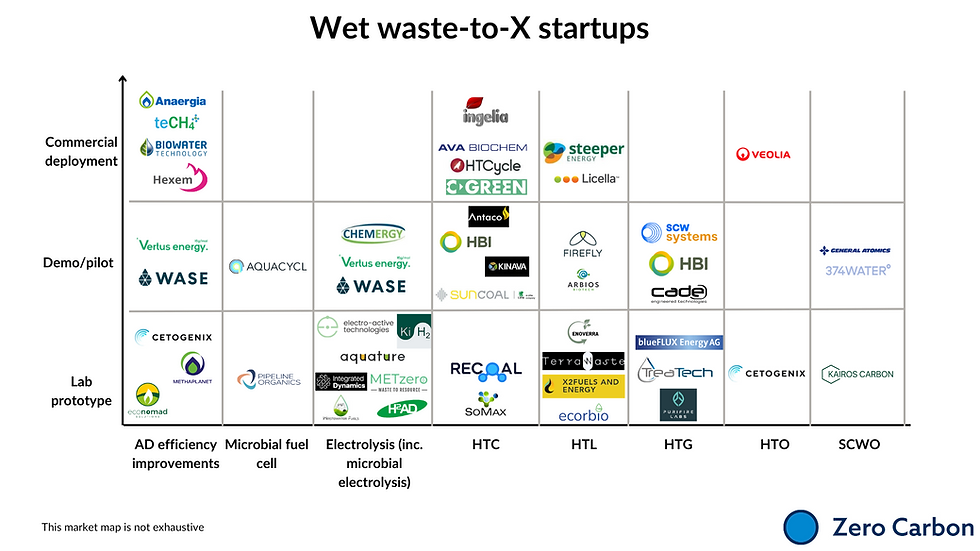
Outlook
Considering wet waste processing for valorisation, anaerobic digestion and hydrothermal carbonisation (and to some extent HTL/HTG) are largely mature technologies. Both produce biosolids and biogas in different amounts. Technologies based on bio-electrochemical processes, and the hydrothermal processes that require more extreme conditions, are more nascent and will require more funding to reach higher TRL levels for commercial deployment.
From a waste management perspective, it is also important to bear in mind:
the value of technologies that reduce waste volumes e.g. minimising biosolids and have additional water treatment co-benefits beyond valorisation
any incoming regulation, that is likely to become stricter on chemical composition of biosolids landspreading
the challenge of selling emerging technologies to utilities whose profits are limited by regulators
From a valorisation perspective, which product/technology to prioritise depends on cost / value of product and value of CDR. As shown in the figure below (Woodall & McCormick (2022)) the relative value of using carbon-based feedstocks for CDR (in this case municipal solid waste, MSW) eventually exceeds the revenue from using it for electricity, or a hybrid of electricity with carbon capture with increasing carbon removal price.
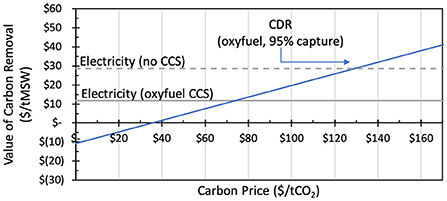
So what technologies do we need? And where do we invest? We know we want to displace fossil fuels for electricity generation, and expect that we will need at least 7 Gt of carbon dioxide removal annually by 2050, so we should try to optimise at the system level, where we can most efficiently meet both of these aims.
Recommended reading
Assessing the optimal uses of biomass: Carbon and energy price conditions for the Aines Principle to apply, C. M. Woodall and C. F. McCormick Front. Clim. 4 (2022)
Bioelectrochemical Treatment Technology—The New Practical Approach for Wastewater Management and GHG Emissions Reduction, S. Babanova et al. Front. Chem. Eng. 4 (2022)
Microbial Electrolysis Cells for Decentralised Wastewater Treatment: The Next Steps, T. Fudge et al. Water 13 (2021)
Resource recovery and waste-to-energy from wastewater sludge via thermochemical conversion technologies in support of circular economy: a comprehensive review, R. R. Bora, R. E. Richardson and F. You, BMC Chem. Eng. 2 (2020)
Outlook for biogas and biomethane: Prospects for organic growth International Energy Agency (2020)
BECCS Vs BiCRS: Should Biomass Be Used Primarily As An Energy Source Or As A Route For Carbon Removal? Poppy Russell, Counteract (2022)
What's the best use for our 'waste' biomass? Andy Lubershane, Steel For Fuel (2023)
Acknowledgements
Thank you to those who gave me their time and shared their insights on this topic including Poppy Russell (Counteract), Gabriel Méhaignerie (Pace Ventures), Manuela Cunha Brito (Mudcake), Dan Murray and Megha Raghavan (Kairos Carbon), Danny Bogar and David (374Water), Benjamin Howard (Vertus Energy), Risei Goto (AP Ventures), Mariano Lazarte (Royal Unibrew), Prof Tom Stephenson (Cranfield University)
Comments